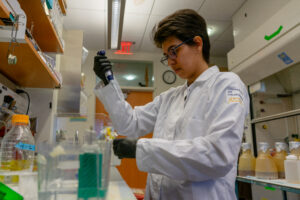
The goal of the Biophysics Graduate Program at Berkeley is to train students to bring a physical approach to the study of biological processes that range in scale from the sub-molecular (studying the interplay of the inter-atomic forces that give proteins their particular shape, motion and function) to the systems level (studying the concerted activity of neural and genetic circuits).
Study in these research areas employs unique types of physical instrumentation and research methodologies such as X-ray diffraction, NMR, EPR and fluorescence spectroscopy, electronic and optical instrumentation, mathematical computer modeling and analysis. The Ph.D. program in biophysics at Berkeley provides a route for students from the physical and biological sciences to enter the leading edge of modern biology with the tools that will permit them to ask the next generation of biological questions. Students admitted to this program have backgrounds in either the physical or biological sciences. In some cases, students have an undergraduate degree in physics, chemistry, engineering, mathematics, or computer science, and may have limited coursework in biology, but have an interest in applying physical and computational approaches to biology. In other cases, students have an undergraduate degree in the biological sciences, with an interest in bringing a quantitative approach to their research.
For information about the research performed in particular laboratories, please see the faculty page.
The research falls into a number of broad categories, links below:
We can infer much about how a neural system functions by quantifying its output (behavior) in relation to its input (stimuli). But to completely understand a neural system, one must also have methods to measure its neural activity. Most such methods are invasive. However, invasive methods (1) alter the system that it sets out to measure, (2) often involve anesthesia that alters neural activity, (3) may inflict suffering on animals, and (4) usually can’t be used to study humans. Non-invasive functional neuro-imaging, although lagging behind invasive methods in spatial and temporal resolution, are mostly devoid of these drawbacks, and therefore are extremely valuable in the study of neural systems.
Members of the Biophysics Group are involved in all aspects of development and practice in this exciting field. Groups with expertise in physics and chemistry are developing the technological hardware and software for methods such as electroencephalography (EEG), functional magnetic resonance imaging (fMRI), and positron emission tomography (PET). Others with expertise in computational modeling are developing methods of analysis for the large and complex data sets generated by neuro-imaging methods. Finally, we are applying these methods to study neural systems in sensory and cognitive processing in both animals and humans, and in both health and disease. In addition to imaging neural activity, these projects often entail efforts to develop new bioelectronics needed for measuring and modifying neural activity. One example is transmagnetic stimulation (TMS), being developed in several Berkeley labs. TMS may be used to generate neural activity de novo in humans, both to probe neural coding and as an option for treating neurological disease. This interdisciplinary combination of physical methods and biological goals puts this research squarely within the purview of the Biophysics Group.
Cell signaling and cell physiology are areas of research that are gaining prominence due to rapid advances in biophysical methods and the integration of these methods with molecular biology, biochemistry and genetics. These advances have allowed studies of molecular processes in the context of the integrated behavior of cells and their organelles, and of tissues and organs, and whole organisms. In addition, the application of theoretical mathematical models to this research is providing unique insights into how the ensemble behaviors of ion channels, pumps and cellular signaling systems determine cell behavior, and how groups of cells cooperatively determine the behavior of complex tissues such as the brain.
Current research in biophysics at Berkeley includes the study of the function and regulation of ion channels and transporters, the protein signaling pathways that regulate cell division and growth, the mechanisms of muscle contraction, nerve secretion, and synaptic plasticity, and the membrane dynamics and trafficking that underlies secretion, membrane wound healing, and signaling by organelles. A strength of the Biophysics Group is in the development and application of novel optical and chemical methods for making real-time high spatial resolution measurements of protein activity and second messenger levels in specific subcellular compartments of cells in intact tissue. Research is focused on both the elucidation of biological mechanisms and the development of genetic therapies for human diseases such as cystic fibrosis, muscular dystrophy, retinitis pigmentosa and macular degeneration in the retina. Promising advances in synthetic biology are also being made here at Berkeley as knowledge of cell signaling and physiology grows.
We study the physics of how organisms perform mechanical functions such as locomotion, feeding, or withstanding environmental forces. We work at the interface between biomechanics and comparative physiology, ecology, and evolutionary biology, and we conduct our research both in the laboratory and in the field within the natural habitats of the organisms. Experimental and computational techniques from both solid and fluid mechanics are combined to study a variety of problems, including animal locomotion in water, on the ground, and in the air, food capture, chemoreception, and the interplay between mechanical design, neural control, and behavioral responses to physically variable environments. Structure and function of diverse plant and animal taxa are investigated at multiple levels of organization, including tissues, organs and organ systems (e.g., legs, wings, circulatory systems, olfactory organs), whole organisms and their interactions with the physical environment, and biomechanical adaptations over evolutionary time. Physical principles learned in our studies of the biomechanics of organisms are providing inspiration for the design of man-made materials and machines (i.e., biomimetics).
By enumerating complete gene repertoires, genomes provide an unprecedented unbiased view of biology. Efforts at Berkeley range from work at Lawrence Berkeley National Laboratory and the Joint Genomics Institute to sequence, catalog, and analyze the genomes of new and model organisms, to theoretical and experimental work to understand genetic circuits. Computational methods are used to discover previously undetected molecular and cellular biological activities, to design computational screens for genetic control elements, and to provide a detailed physical analysis of the biochemical and genetic networks that govern cellular development with the goal of engineering cell and tissue systems.
Computational approaches at Berkeley are also being used to determine the rules of protein folding, in protein design (with an emphasis on enzymes), to understand the dynamics of signaling networks responsible for cell differentiation and motility, to model the energetics of force generation and structural rearrangement in motor proteins, ion channels and pumps, to model population/disease dynamics, and to model information processing by neural networks in vision, audition and learning, and to design systems for computer vision.
Several faculty in the program are members of the Designated Emphasis in Computational and Genomic Biology. This Designated Emphasis (DE) program functions essentially like a “minor,” and provides specialized multi-disciplinary training and research opportunities in the different facets of computational biology and genomics. Biophysics students who wish to follow research careers in genomics or computational biology are encouraged to apply to the DE.
Another large area of active research in the Biophysics Group is molecular microscopy. At the heart of the initiative is the idea that the next generation of microscopy will depend on novel optical probes made of proteins and nucleic acids, rather than organic chemicals. The ability to visualize the targeting within cells of native macromolecules has already become key to understanding their biological function. The next step is to understand how they assemble into the complexes that carry out cellular functions, and to monitor their activities in real-time in vivo with microscopy so that the spatial location of the activity can be understood.
Fluorescent indicator dyes have revolutionized our understanding of cellular signaling by providing continuous measurements of physiological events in single cells and cell populations with high temporal and spatial resolution. Until now, these organic dyes have been limited in scope because they must be synthesized chemically and introduced as hydrolyzable esters or by microinjection, meaning that they either fill all cells in a tissue entirely, or that only one or a small number of cells are labeled. In absence of targeting of the organic dyes to specific cell types, optical signals of interest are often drowned out either by indicator background from inactive cells, or by signals in cells that are not the focus of interest. Moreover, local signals due to protein-protein interactions are not measurable in the bulk cytoplasm, which organic dyes fill.
Studies relating the structure and dynamics of biological macromolecules to function are a significant part of modern biophysics. This area of research spans a large range of topics and is well represented in the Biophysics Graduate Group at Berkeley. Current areas of research include how soluble proteins involved in signaling, regulation, and key enzymatic steps interact to carry out their function, and how membrane proteins change conformation to generate signals, pump ions and molecules or to affect membrane fusion. Studies focus on the structure of proteins, RNA and DNA with an emphasis on the rules and trajectories of protein folding, the rapid protein motions of channels, pumps and motors, photochemistry and phototransduction, the molecular interactions between proteins and DNA, the mechanism of RNA catalysis, and the design of ligands that bind to nucleic acids and act as specific regulators.
Insights into biomolecular behavior are leading to practical applications in biotechnology and bioengineering. The complementary skills of scientists in biology, physics, chemistry and engineering in approaching these areas of research enhances progress tremendously.
A major strength at Berkeley is the wide range of facilities and technologies available for the study of macromolecules. For X-ray diffraction studies, the Advanced Light Source (ALS) at Lawrence Berkeley National Laboratory has stunning capabilities. Also housed here is one of the best cryo electron microscopes available for electron microscopy. Modern instruments for magnetic resonance spectroscopy (both NMR and EPR) can provide structural insights, and are also unparalleled for probing dynamics. Recent methods in mass spectrometry are also being applied at several of the outstanding on-campus facilities (which includes the national HHMI mass spectrometry laboratory). To resolve ultra-fast processes, optical, Raman, and fluorescence spectroscopies with femtosecond pulsed laser systems are used. Single molecules can now be observed or manipulated with atomic force microscopy, optical tweezers, fluorescence probes or single-channel electrical measurements. Theory and modeling are critical parts of testing the ideas generated by new experiments, and for understanding complex processes. Computer modeling of protein folding is providing insight into features which control folding pathways, as well as the roles of solvent interactions.
Systems neuroscience addresses the function of neural circuits in intact organisms. The ultimate goal of this area of research is to understand the function of the nervous system at a variety of levels, from single cells to entire networks that mediate complex behaviors such as vision, audition and motor responses. Because systems neuroscience addresses such a broad range of issues, it integrates experimental, analytic and theoretical techniques from a wide range of disciplines. In particular many important analytic and theoretical approaches were developed first in physics and engineering and have been modified for application to neurobiology. At the finest scale of analysis, systems researchers investigate how single cells function as computational units. These studies assess the roles of channel distribution, electrical cable properties and dendritic architecture in neural computation.
Many systems researchers are particularly interested in the biophysical mechanisms that confer important computational properties such as stimulus selectivity, adaptation and learning in single cells. At a more abstract level, systems neuroscience considers how action potentials encode information about the world, and how information can be represented in a network of many cells. Research in this area was made possible by the development of information theory in engineering and physics. At the largest scale, systems neuroscientists investigate the way distinct cortical regions represent information about the world, and how these areas work together to produce integrated behavior. Much of our understanding of representational issues originated in computer science and robotics, while both experimental and analytic approaches to cortical integration are based on principles first developed in physics.
A major focus of research in this area is on visual processing, from retinal phototransduction, connectivity and signal processing, to the response properties and information transfer in the thalamus and primary visual areas of the cortex, to higher areas of the brain. Considerable effort is focused on understanding the visual processing of natural scenes, the role of attention, and the segmentation of visual information. Other areas of research include auditory processing, the memory basis of vocal learning, and olfactory processing.